Fatty acid biosynthesis revisited: structure elucidation and metabolic engineering
- PMID: 25360565
- PMCID: PMC4276719
- DOI: 10.1039/c4mb00443d
Fatty acid biosynthesis revisited: structure elucidation and metabolic engineering
Abstract
Fatty acids are primary metabolites synthesized by complex, elegant, and essential biosynthetic machinery. Fatty acid synthases resemble an iterative assembly line, with an acyl carrier protein conveying the growing fatty acid to necessary enzymatic domains for modification. Each catalytic domain is a unique enzyme spanning a wide range of folds and structures. Although they harbor the same enzymatic activities, two different types of fatty acid synthase architectures are observed in nature. During recent years, strained petroleum supplies have driven interest in engineering organisms to either produce more fatty acids or specific high value products. Such efforts require a fundamental understanding of the enzymatic activities and regulation of fatty acid synthases. Despite more than one hundred years of research, we continue to learn new lessons about fatty acid synthases' many intricate structural and regulatory elements. In this review, we summarize each enzymatic domain and discuss efforts to engineer fatty acid synthases, providing some clues to important challenges and opportunities in the field.
Figures
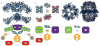
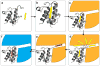
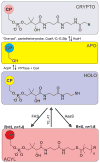
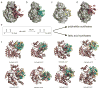
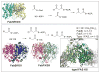
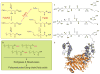
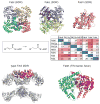
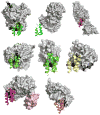
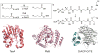
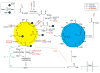
Similar articles
-
The structural biology of type II fatty acid biosynthesis.Annu Rev Biochem. 2005;74:791-831. doi: 10.1146/annurev.biochem.74.082803.133524. Annu Rev Biochem. 2005. PMID: 15952903 Review.
-
Sunflower (Helianthus annuus) fatty acid synthase complex: enoyl-[acyl carrier protein]-reductase genes.Planta. 2015 Jan;241(1):43-56. doi: 10.1007/s00425-014-2162-7. Epub 2014 Sep 11. Planta. 2015. PMID: 25204631
-
Cloning, expression, characterization, and interaction of two components of a human mitochondrial fatty acid synthase. Malonyltransferase and acyl carrier protein.J Biol Chem. 2003 Oct 10;278(41):40067-74. doi: 10.1074/jbc.M306121200. Epub 2003 Jul 25. J Biol Chem. 2003. PMID: 12882974
-
Discovery of a Regulatory Subunit of the Yeast Fatty Acid Synthase.Cell. 2020 Mar 19;180(6):1130-1143.e20. doi: 10.1016/j.cell.2020.02.034. Epub 2020 Mar 10. Cell. 2020. PMID: 32160528
-
Using modern tools to probe the structure-function relationship of fatty acid synthases.Chembiochem. 2015 Mar 2;16(4):528-547. doi: 10.1002/cbic.201402578. Epub 2015 Feb 10. Chembiochem. 2015. PMID: 25676190 Free PMC article. Review.
Cited by
-
Enhancing isoprenol production by systematically tuning metabolic pathways using CRISPR interference in E. coli.Front Bioeng Biotechnol. 2023 Nov 6;11:1296132. doi: 10.3389/fbioe.2023.1296132. eCollection 2023. Front Bioeng Biotechnol. 2023. PMID: 38026852 Free PMC article.
-
Extracellular Lipids in the Lung and Their Role in Pulmonary Fibrosis.Cells. 2022 Apr 3;11(7):1209. doi: 10.3390/cells11071209. Cells. 2022. PMID: 35406772 Free PMC article. Review.
-
Genomic, Transcriptomic, and Epigenomic Features Differentiate Genes That Are Relevant for Muscular Polyunsaturated Fatty Acids in the Common Carp.Front Genet. 2019 Mar 15;10:217. doi: 10.3389/fgene.2019.00217. eCollection 2019. Front Genet. 2019. PMID: 30930941 Free PMC article.
-
Active site labeling of fatty acid and polyketide acyl-carrier protein transacylases.Org Biomol Chem. 2019 May 15;17(19):4720-4724. doi: 10.1039/c8ob03229g. Org Biomol Chem. 2019. PMID: 31044196 Free PMC article.
-
Identification of the Candidate Proteins Related to Oleic Acid Accumulation during Peanut (Arachis hypogaea L.) Seed Development through Comparative Proteome Analysis.Int J Mol Sci. 2018 Apr 18;19(4):1235. doi: 10.3390/ijms19041235. Int J Mol Sci. 2018. PMID: 29670063 Free PMC article.
References
Publication types
MeSH terms
Substances
Grants and funding
LinkOut - more resources
Full Text Sources
Other Literature Sources
Molecular Biology Databases