Reversing DNA methylation: mechanisms, genomics, and biological functions
- PMID: 24439369
- PMCID: PMC3938284
- DOI: 10.1016/j.cell.2013.12.019
Reversing DNA methylation: mechanisms, genomics, and biological functions
Abstract
Methylation of cytosines in the mammalian genome represents a key epigenetic modification and is dynamically regulated during development. Compelling evidence now suggests that dynamic regulation of DNA methylation is mainly achieved through a cyclic enzymatic cascade comprised of cytosine methylation, iterative oxidation of methyl group by TET dioxygenases, and restoration of unmodified cytosines by either replication-dependent dilution or DNA glycosylase-initiated base excision repair. In this review, we discuss the mechanism and function of DNA demethylation in mammalian genomes, focusing particularly on how developmental modulation of the cytosine-modifying pathway is coupled to active reversal of DNA methylation in diverse biological processes.
Copyright © 2014 Elsevier Inc. All rights reserved.
Figures
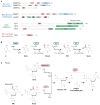
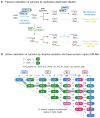
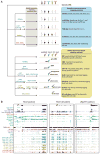
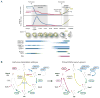
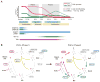
Similar articles
-
TET enzymes, TDG and the dynamics of DNA demethylation.Nature. 2013 Oct 24;502(7472):472-9. doi: 10.1038/nature12750. Nature. 2013. PMID: 24153300 Free PMC article. Review.
-
Tet family of 5-methylcytosine dioxygenases in mammalian development.J Hum Genet. 2013 Jul;58(7):421-7. doi: 10.1038/jhg.2013.63. Epub 2013 May 30. J Hum Genet. 2013. PMID: 23719188 Free PMC article. Review.
-
TET-mediated active DNA demethylation: mechanism, function and beyond.Nat Rev Genet. 2017 Sep;18(9):517-534. doi: 10.1038/nrg.2017.33. Epub 2017 May 30. Nat Rev Genet. 2017. PMID: 28555658 Review.
-
Genome-wide analysis reveals TET- and TDG-dependent 5-methylcytosine oxidation dynamics.Cell. 2013 Apr 25;153(3):692-706. doi: 10.1016/j.cell.2013.04.002. Epub 2013 Apr 18. Cell. 2013. PMID: 23602152 Free PMC article.
-
TET enzymatic oxidation of 5-methylcytosine, 5-hydroxymethylcytosine and 5-formylcytosine.Mutat Res Genet Toxicol Environ Mutagen. 2014 Apr;764-765:18-35. doi: 10.1016/j.mrgentox.2013.09.001. Epub 2013 Sep 14. Mutat Res Genet Toxicol Environ Mutagen. 2014. PMID: 24045206 Review.
Cited by
-
Muscle regeneration controlled by a designated DNA dioxygenase.Cell Death Dis. 2021 May 25;12(6):535. doi: 10.1038/s41419-021-03817-2. Cell Death Dis. 2021. PMID: 34035232 Free PMC article.
-
Epigenetic control of myeloid cell differentiation, identity and function.Nat Rev Immunol. 2015 Jan;15(1):7-17. doi: 10.1038/nri3777. Nat Rev Immunol. 2015. PMID: 25534619 Review.
-
Epigenetic Changes during Hepatic Stellate Cell Activation.PLoS One. 2015 Jun 12;10(6):e0128745. doi: 10.1371/journal.pone.0128745. eCollection 2015. PLoS One. 2015. PMID: 26065684 Free PMC article.
-
TET Enzymes and 5-Hydroxymethylcytosine in Neural Progenitor Cell Biology and Neurodevelopment.Front Cell Dev Biol. 2021 Feb 18;9:645335. doi: 10.3389/fcell.2021.645335. eCollection 2021. Front Cell Dev Biol. 2021. PMID: 33681230 Free PMC article. Review.
-
Epigenetic Modification Agents Improve Gene-Specific Methylation Reprogramming in Porcine Cloned Embryos.PLoS One. 2015 Jun 11;10(6):e0129803. doi: 10.1371/journal.pone.0129803. eCollection 2015. PLoS One. 2015. PMID: 26068219 Free PMC article.
References
-
- Barreto G, Schafer A, Marhold J, Stach D, Swaminathan SK, Handa V, Doderlein G, Maltry N, Wu W, Lyko F, et al. Gadd45a promotes epigenetic gene activation by repair-mediated DNA demethylation. Nature. 2007;445:671–675. - PubMed
-
- Bernstein BE, Mikkelsen TS, Xie X, Kamal M, Huebert DJ, Cuff J, Fry B, Meissner A, Wernig M, Plath K, et al. A bivalent chromatin structure marks key developmental genes in embryonic stem cells. Cell. 2006;125:315–326. - PubMed
Publication types
MeSH terms
Substances
Grants and funding
LinkOut - more resources
Full Text Sources
Other Literature Sources