Nonselective Wiring Accounts for Red-Green Opponency in Midget Ganglion Cells of the Primate Retina
- PMID: 29305531
- PMCID: PMC5815352
- DOI: 10.1523/JNEUROSCI.1688-17.2017
Nonselective Wiring Accounts for Red-Green Opponency in Midget Ganglion Cells of the Primate Retina
Abstract
In primate retina, "red-green" color coding is initiated when signals originating in long (L) and middle (M) wavelength-sensitive cone photoreceptors interact antagonistically. The center-surround receptive field of "midget" ganglion cells provides the neural substrate for L versus M cone-opponent interaction, but the underlying circuitry remains unsettled, centering around the longstanding question of whether specialized cone wiring is present. To address this question, we measured the strength, sign, and spatial tuning of L- and M-cone input to midget receptive fields in the peripheral retina of macaque primates of either sex. Consistent with previous work, cone opponency arose when one of the cone types showed a stronger connection to the receptive field center than to the surround. We implemented a difference-of-Gaussians spatial receptive field model, incorporating known biology of the midget circuit, to test whether physiological responses we observed in real cells could be captured entirely by anatomical nonselectivity. When this model sampled nonselectively from a realistic cone mosaic, it accurately reproduced key features of a cone-opponent receptive field structure, and predicted both the variability and strength of cone opponency across the retina. The model introduced here is consistent with abundant anatomical evidence for nonselective wiring, explains both local and global properties of the midget population, and supports a role in their multiplexing of spatial and color information. It provides a neural basis for human chromatic sensitivity across the visual field, as well as the maintenance of normal color vision despite significant variability in the relative number of L and M cones across individuals.SIGNIFICANCE STATEMENT Red-green color vision is a hallmark of the human and nonhuman primate that starts in the retina with the presence of long (L)- and middle (M)-wavelength sensitive cone photoreceptor types. Understanding the underlying retinal mechanism for color opponency has focused on the broad question of whether this characteristic can emerge from nonselective wiring, or whether complex cone-type-specific wiring must be invoked. We provide experimental and modeling support for the hypothesis that nonselective connectivity is sufficient to produce the range of red-green color opponency observed in midget ganglion cells across the retina. Our nonselective model reproduces the diversity of physiological responses of midget cells while also accounting for systematic changes in color sensitivity across the visual field.
Keywords: circuitry; color; ganglion cell; primate; retina; vision.
Copyright © 2018 the authors 0270-6474/18/381520-21$15.00/0.
Figures
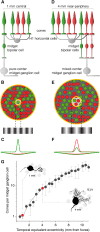
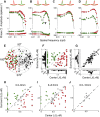
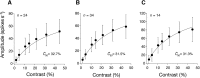
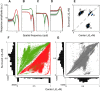
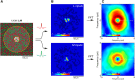
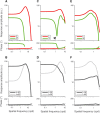
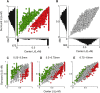
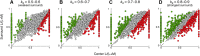
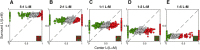
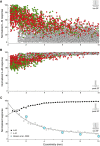
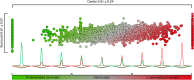
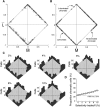
Similar articles
-
Connectomic Identification and Three-Dimensional Color Tuning of S-OFF Midget Ganglion Cells in the Primate Retina.J Neurosci. 2019 Oct 2;39(40):7893-7909. doi: 10.1523/JNEUROSCI.0778-19.2019. Epub 2019 Aug 12. J Neurosci. 2019. PMID: 31405926 Free PMC article.
-
L and M cone contributions to the midget and parasol ganglion cell receptive fields of macaque monkey retina.J Neurosci. 2004 Feb 4;24(5):1079-88. doi: 10.1523/JNEUROSCI.3828-03.2004. J Neurosci. 2004. PMID: 14762126 Free PMC article.
-
Horizontal cell feedback without cone type-selective inhibition mediates "red-green" color opponency in midget ganglion cells of the primate retina.J Neurosci. 2011 Feb 2;31(5):1762-72. doi: 10.1523/JNEUROSCI.4385-10.2011. J Neurosci. 2011. PMID: 21289186 Free PMC article.
-
Circuitry for color coding in the primate retina.Proc Natl Acad Sci U S A. 1996 Jan 23;93(2):582-8. doi: 10.1073/pnas.93.2.582. Proc Natl Acad Sci U S A. 1996. PMID: 8570599 Free PMC article. Review.
-
Distinct synaptic mechanisms create parallel S-ON and S-OFF color opponent pathways in the primate retina.Vis Neurosci. 2014 Mar;31(2):139-51. doi: 10.1017/S0952523813000230. Epub 2013 Jul 29. Vis Neurosci. 2014. PMID: 23895762 Free PMC article. Review.
Cited by
-
A circuit motif for color in the human foveal retina.Proc Natl Acad Sci U S A. 2024 Sep 3;121(36):e2405138121. doi: 10.1073/pnas.2405138121. Epub 2024 Aug 27. Proc Natl Acad Sci U S A. 2024. PMID: 39190352 Free PMC article.
-
An S-cone circuit for edge detection in the primate retina.Sci Rep. 2019 Aug 15;9(1):11913. doi: 10.1038/s41598-019-48042-2. Sci Rep. 2019. PMID: 31417169 Free PMC article.
-
Reconciling Color Vision Models With Midget Ganglion Cell Receptive Fields.Front Neurosci. 2019 Aug 16;13:865. doi: 10.3389/fnins.2019.00865. eCollection 2019. Front Neurosci. 2019. PMID: 31474825 Free PMC article. Review.
-
Effect of cone spectral topography on chromatic detection sensitivity.J Opt Soc Am A Opt Image Sci Vis. 2020 Apr 1;37(4):A244-A254. doi: 10.1364/JOSAA.382384. J Opt Soc Am A Opt Image Sci Vis. 2020. PMID: 32400553 Free PMC article.
-
Gaining the system: limits to compensating color deficiencies through post-receptoral gain changes.J Opt Soc Am A Opt Image Sci Vis. 2023 Mar 1;40(3):A16-A25. doi: 10.1364/JOSAA.480035. J Opt Soc Am A Opt Image Sci Vis. 2023. PMID: 37132998 Free PMC article.
References
Publication types
MeSH terms
Grants and funding
LinkOut - more resources
Full Text Sources
Other Literature Sources