Unconventional van der Waals heterostructures beyond stacking
- PMID: 34553135
- PMCID: PMC8441167
- DOI: 10.1016/j.isci.2021.103050
Unconventional van der Waals heterostructures beyond stacking
Abstract
Two-dimensional crystals provide exceptional opportunities for integrating dissimilar materials and forming interfaces where distinct properties and phenomena emerge. To date, research has focused on two basic heterostructure types: vertical van der Waals stacks and laterally joined monolayer crystals with in-plane line interfaces. Much more diverse architectures and interface configurations can be realized in the few-layer and multilayer regime, and if mechanical stacking and single-layer growth are replaced by processes taking advantage of self-organization, conversions between polymorphs, phase separation, strain effects, and shaping into the third dimension. Here, we highlight such opportunities for engineering heterostructures, focusing on group IV chalcogenides, a class of layered semiconductors that lend themselves exceptionally well for exploring novel van der Waals architectures, as well as advanced methods including in situ microscopy during growth and nanometer-scale probes of light-matter interactions. The chosen examples point to fruitful future directions and inspire innovative developments to create unconventional van der Waals heterostructures beyond stacking.
Keywords: materials synthesis; nanotechnology; nanotechnology fabrication.
© 2021 The Authors.
Conflict of interest statement
The authors declare no competing interests.
Figures
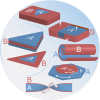
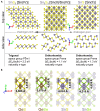
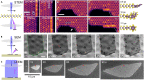
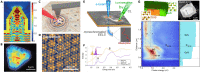
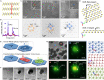
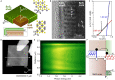
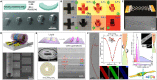
Similar articles
-
Lateral Heterostructures of Multilayer GeS and SnS van der Waals Crystals.ACS Nano. 2020 Sep 22;14(9):12248-12255. doi: 10.1021/acsnano.0c05978. Epub 2020 Sep 4. ACS Nano. 2020. PMID: 32886477
-
Multilayer Lateral Heterostructures of Van Der Waals Crystals with Sharp, Carrier-Transparent Interfaces.Adv Sci (Weinh). 2022 Jan;9(3):e2103830. doi: 10.1002/advs.202103830. Epub 2021 Nov 23. Adv Sci (Weinh). 2022. PMID: 34813175 Free PMC article.
-
Photonics in Multimaterial Lateral Heterostructures Combining Group IV Chalcogenide van der Waals Semiconductors.Small. 2024 May;20(20):e2307372. doi: 10.1002/smll.202307372. Epub 2023 Dec 6. Small. 2024. PMID: 38054819
-
Two-Dimensional van der Waals Superconductor Heterostructures: Josephson Junctions and Beyond.Precis Chem. 2024 Mar 12;2(7):273-281. doi: 10.1021/prechem.3c00126. eCollection 2024 Jul 22. Precis Chem. 2024. PMID: 39473900 Free PMC article. Review.
-
Van der Waals Heterostructures for High-Performance Device Applications: Challenges and Opportunities.Adv Mater. 2020 Jul;32(27):e1903800. doi: 10.1002/adma.201903800. Epub 2019 Oct 14. Adv Mater. 2020. PMID: 31608514 Review.
Cited by
-
Evaluating the resolution of conventional optical microscopes through point spread function measurement.iScience. 2023 Sep 21;26(10):107976. doi: 10.1016/j.isci.2023.107976. eCollection 2023 Oct 20. iScience. 2023. PMID: 37822495 Free PMC article. Review.
References
-
- Agnoli S., Granozzi G. Second generation graphene: opportunities and challenges for surface science. Surf. Sci. 2013;609:1–5. doi: 10.1016/j.susc.2012.11.016. - DOI
Publication types
LinkOut - more resources
Full Text Sources