A Human Brain-Chip for Modeling Brain Pathologies and Screening Blood-Brain Barrier Crossing Therapeutic Strategies
- PMID: 39458643
- PMCID: PMC11510380
- DOI: 10.3390/pharmaceutics16101314
A Human Brain-Chip for Modeling Brain Pathologies and Screening Blood-Brain Barrier Crossing Therapeutic Strategies
Abstract
Background/Objectives: The limited translatability of preclinical experimental findings to patients remains an obstacle for successful treatment of brain diseases. Relevant models to elucidate mechanisms behind brain pathogenesis, including cell-specific contributions and cell-cell interactions, and support successful _targeting and prediction of drug responses in humans are urgently needed, given the species differences in brain and blood-brain barrier (BBB) functions. Human microphysiological systems (MPS), such as Organ-Chips, are emerging as a promising approach to address these challenges. Here, we examined and advanced a Brain-Chip that recapitulates aspects of the human cortical parenchyma and the BBB in one model. Methods: We utilized human primary astrocytes and pericytes, human induced pluripotent stem cell (hiPSC)-derived cortical neurons, and hiPSC-derived brain microvascular endothelial-like cells and included for the first time on-chip hiPSC-derived microglia. Results: Using Tumor necrosis factor alpha (TNFα) to emulate neuroinflammation, we demonstrate that our model recapitulates in vivo-relevant responses. Importantly, we show microglia-derived responses, highlighting the Brain-Chip's sensitivity to capture cell-specific contributions in human disease-associated pathology. We then tested BBB crossing of human transferrin receptor antibodies and conjugated adeno-associated viruses. We demonstrate successful in vitro/in vivo correlation in identifying crossing differences, underscoring the model's capacity as a screening platform for BBB crossing therapeutic strategies and ability to predict in vivo responses. Conclusions: These findings highlight the potential of the Brain-Chip as a reliable and time-efficient model to support therapeutic development and provide mechanistic insights into brain diseases, adding to the growing evidence supporting the value of MPS in translational research and drug discovery.
Keywords: cell-specific contributions; microfluidics brain-chip; physiologically relevant responses; screening of BBB-crossing therapeutics.
Conflict of interest statement
S.M.C., K.H. and B.Z. are full-time employees of Regeneron Pharmaceuticals Inc. and receive stock options/restricted stock as part of their compensation. K.K. and E.P. were full-time employees of Regeneron Pharmaceuticals Inc. and received stock options/restricted stock as part of their compensation during their employment.
Figures
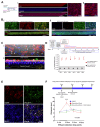
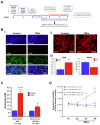
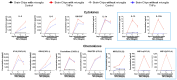
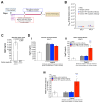
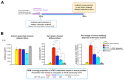
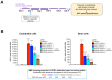
Similar articles
-
A perfused human blood-brain barrier on-a-chip for high-throughput assessment of barrier function and antibody transport.Fluids Barriers CNS. 2018 Aug 31;15(1):23. doi: 10.1186/s12987-018-0108-3. Fluids Barriers CNS. 2018. PMID: 30165870 Free PMC article.
-
Aptamer Nanoconstructs Crossing Human Blood-Brain Barrier Discovered via Microphysiological System-Based SELEX Technology.ACS Nano. 2023 May 9;17(9):8153-8166. doi: 10.1021/acsnano.2c11675. Epub 2023 Apr 17. ACS Nano. 2023. PMID: 37068137
-
Human iPSC-Derived Blood-Brain Barrier Chips Enable Disease Modeling and Personalized Medicine Applications.Cell Stem Cell. 2019 Jun 6;24(6):995-1005.e6. doi: 10.1016/j.stem.2019.05.011. Cell Stem Cell. 2019. PMID: 31173718
-
Engineering Brain-Specific Pericytes from Human Pluripotent Stem Cells.Tissue Eng Part B Rev. 2020 Aug;26(4):367-382. doi: 10.1089/ten.TEB.2020.0091. Tissue Eng Part B Rev. 2020. PMID: 32571167 Free PMC article. Review.
-
Recent advances in human iPSC-derived models of the blood-brain barrier.Fluids Barriers CNS. 2020 Apr 22;17(1):30. doi: 10.1186/s12987-020-00191-7. Fluids Barriers CNS. 2020. PMID: 32321511 Free PMC article. Review.
References
-
- Atkins J.T., George G.C., Hess K., Marcelo-Lewis K.L., Yuan Y., Borthakur G., Khozin S., LoRusso P., Hong D.S. Pre-clinical animal models are poor predictors of human toxicities in phase 1 oncology clinical trials. Br. J. Cancer. 2020;123:1496–1501. doi: 10.1038/s41416-020-01033-x. - DOI - PMC - PubMed
Grants and funding
LinkOut - more resources
Full Text Sources